Enzymes are biocatalysts that catalyse biochemical reactions. They help to lower the activation energy of a reaction by binding to a substrate and holding it there so the reaction can occur.
They are large proteins that can also be found in RNA form (catalytic RNAs or ribozymes). They aid in a variety of bodily functions, including growth and development, reproduction, and numerous life processes.
Important factors in enzyme structure
Enzymes are extremely specific to their substrate and have high catalytic power, which means they can significantly increase the reaction pace without changing the substrate. The activity, functioning, and potential of an enzyme can be affected by various circumstances, including chemical and physical ones. The enzymatic activity of proteinous enzymes may be affected depending on the conformational structure and its denaturation.
The catalytic process carried out by enzymes occurs at a specific location on the enzyme. This area is known as the active site, and it accounts for only a small portion of the enzyme’s overall size. The active site is made up of polypeptide chain segments, the active site has a three-dimensional structure.
The majority of enzymes are proteins with catalytic properties required to carry out various operations. Because of their importance in supporting life processes, enzyme regulation has long been a significant component in clinical diagnosis. They are used as biological markers (alteration in a measurable medium such as tissue fluid) to diagnose diseases such as cancer and infarction.
An enzyme’s catalytic cycle is explained below:
At first, the substrate binds to the enzyme’s active site, fitting into the active site. The enzyme’s shape changes as a result of the substrate’s binding, causing it to fit more tightly around the substrate (induced fit).
The enzyme’s active site, which is now close to the substrate, breaks the substrate’s chemical bonds, forming a new enzyme-product complex.
Lastly, the reaction products are released by the enzyme, and the free enzyme is ready to attach to another molecule of the substrate, and this catalytic cycle is repeated again.
Combining of the enzyme (E) and the substrate (S) so that a highly reactive enzyme-substrate complex (ES) is produced.
The disintegration of the complex molecule to give the enzyme-product complex (EP).
Thus, the whole catalyst action of enzymes is summarised as:
Fischer’s Lock and Key model
In 1894, Emil Fischer introduced the Lock and Key Model to propose the Lock and Key analogy to illustrate the unique action of an enzyme with a single substrate. The enzyme is the lock, and the substrate is the key. Only the right-sized key (substrate) fits through the lock’s keyhole (active site of the enzyme).
Smaller keys, larger keys, or teeth on keys that are wrongly positioned (substrate molecules incorrectly formed or sized) do not fit into the lock (enzyme). A key with the correct shape can only open a specific lock.
Induced Fit Theory
For many years, scientists thought that enzyme-substrate binding took place in a simple “lock-and-key” fashion. Daniel Koshland suggested the induced fit model in 1958. It is the more accepted model for enzyme-substrate complex than the lock-and-key model.
This theorises that the initial connection between enzyme and substrate is weak, but these weak interactions cause rapid conformational changes in the enzyme, resulting in a stronger binding. In other words, as the enzyme and substrate contact, a minor alteration in the enzyme’s structure occurs, confirming a perfect binding arrangement between the enzyme and the substrate. This dynamic binding enhances the enzyme’s ability to catalyse its reaction.
Factors affecting enzyme activity
The activity of an enzyme can be affected by its environmental factors. Temperature, pH, substrate concentration, and modulators are essential parameters that influence the rate of enzyme-catalysed reactions.
Enzyme concentration
The activity of an enzyme increases as the concentration of the enzyme increases. This is because more enzymes are available to bind to the substrate. In turn, the reaction speed increases.
As long as there is a substrate to bind to, increasing enzyme concentration will speed up the reaction.
The reaction will no longer speed up once all of the substrates have been bound to the enzyme, as there will be nothing for the new enzymes to bind to.
- An increase in enzyme concentration will increase the reaction rate until it reaches a certain point, after which it will remain constant. The number of active sites available increases as the enzyme concentration rises.
The rate of reaction is proportional to the amount of enzyme present. Therefore, we see a straight line in the graph, where the x-axis is enzyme concentration, and the y-axis is the rate of reaction.
Substrate concentration
An enzyme’s activity increases with the rise in substrate concentration. The enzyme activity rises until it reaches a maximum limit.
In other words, the enzyme molecules are completely saturated with the substrate. This means that all enzymes’ active sites are occupied. The surplus substrate molecules will not react until the substrate that has already been bound to the enzymes has reacted and has been released or released without reacting.
To help you visualise, the rate of reaction increases initially. However, the reaction rate reaches a plateau when all enzymes are occupied. For an enzyme-catalysed reaction, there is usually a hyperbolic relationship between the reaction rate and the substrate concentration.
Effect of pH on the rate of reaction
pH has an impact on enzyme activity. A bell-shaped curve emerges when enzyme activity is plotted versus pH. Each enzyme has a certain optimal pH at which the reaction rate is the fastest. The optimal pH is when a specific enzyme’s activity is at its peak. The enzyme activity is greatly reduced below and above the optimal pH, and at high pH, the enzyme becomes completely inactive.
Acidic carboxylic groups (COOH–) and basic amino groups () are found in enzymes. As a result, changing the pH value affects the enzymes.
For most enzymes, the optimum pH for the enzymatic activity of ranges from 6 to 7.
The salivary amylase enzyme is most active at pH 6.8. Amylase is primarily produced by the salivary glands and the pancreas in the human body. The reaction rate decreases, and the enzymes denatured above and below this optimal pH range. At pH 6.8, the enzyme salivary amylase is most active. Because of the high acidity in our stomachs, salivary amylase denatures and changes form. As a result, once the salivary amylase reaches the stomach, it ceases to function.
However, there are a few exceptions.
Pepsin’s optimal pH is 1.8. Pepsin is the primary enzyme found in gastric juice. Lipases, amylases, and proteases are secreted from the pancreas into the small intestine in response to food ingestion. These enzymes are responsible for most nutrient digestion. Pepsin acts best at 1.8 pH because the carboxylic acid group on the amino acid in the enzyme’s active site must be protonated or attached to a hydrogen atom. The carboxylic acid group is protonated at low pH, which allows pepsin to catalyse the chemical reaction of breaking chemical bonds.
Trysin’s optimum pH is 9. Trypsin is an enzyme that aids in protein digestion. Trypsin breaks down proteins in the small intestine, continuing the digesting process that began in the stomach. It is also known as a proteinase or a proteolytic enzyme. The pancreas produces trypsinogen, which is an inactive version of trypsin.
Effects of temperature on the rate of reaction
An optimum temperature range is required to maximise the enzyme activity. Temperatures that are either higher or lower than the optimal temperature causes a decrease in the enzyme activity and can lead to denaturation (when the temperature is too high).
The temperatures between 37 and 45 are referred to as the optimal temperature for most enzymes. At this given temperature, most enzymes become more active. Extremely high temperatures can cause an enzyme to lose its denature form and eventually stop functioning. The majority of enzymes in the human body have a temperature optimum of 37 °C and are denatured or destroyed at higher temperatures.
However, there are some exceptions, for example, in extremophiles. Few enzymes, such as Taq DNA polymerase found in thermophilic bacteria, are active at temperatures as high as 100°C.
Extremophiles are organisms that live in environments with extreme conditions, such as deserts and hydrothermal vents.
Some of these extremophiles contain hyperthermophilic enzymes. Hyperthermophilic enzymes are thermostable (i.e., resistant to irreversible inactivation at high temperatures) and ideally active at high temperatures. Hyperthermophiles generate them (bacteria and archaea with optimal growth temperatures of >80°C).
Enzyme inhibitors and activators
Activators (also known as co-enzymes), such as metal ions, are required for optimal enzyme activity.
The chloride ion, for example, is required for amylase to operate. Amylase is a digestive enzyme secreted by the pancreas and salivary glands also found in other tissues in very small quantities. Metal ions or cofactors, for example, are required to regulate or initiate the catalytic activity of several enzymes.
Enzyme inhibitors can be used as drugs in the treatment of various diseases. Some antimicrobial drugs are enzyme inhibitors that deactivate the enzymes that are needed for the survival of pathogens.
Depending on the inhibition kinetics, enzyme inhibition can be classified as reversible or irreversible inhibition.
Irreversible inhibitors
Irreversible inhibitors bind to an enzyme in a strong covalent connection. These inhibitors might act on the active site, close to it, or far away. As a result, adding more substrate may not be enough to eliminate them. In either event, the enzyme’s basic structure is altered to the point that it no longer functions.
In simple words, the inhibitor binds very securely to the enzyme and does not dissociate from it.
Penicillin, for example, serves as an inhibitor by binding to the enzyme transpeptidase, which is responsible for bacterial cell wall formation. As a result, the drug’s interaction with the enzyme stops cell wall formation, killing the bacteria.
Reversible inhibitors
In reversible inhibition, the inhibitor rapidly dissociates from the enzyme-inhibitor complex. There are three types of reversible inhibitions: competitive, non-competitive and uncompetitive (anti-competitive).
Competitive inhibition
When an inhibitor and a substrate bind to the enzyme in a competitive way, this is known as competitive inhibition. Any molecule with a chemical structure and molecular geometry similar to the substrate could be used as a competitive inhibitor. Contacts between the inhibitor and the enzyme in the active site may be strong. Still, no reaction will occur since the inhibitor does not have the same chemical reactivity as the enzyme, eventually lowering the reaction rate. Competitive inhibition is usually temporary and reversible.
Sulfanilamide competitively binds to the enzyme in the dihydropteroate synthase (DHPS) active site by mimicking para-aminobenzoic acid (PABA) substrate. This prevents the substrate from binding, which halts the production of folic acid, an essential nutrient. Bacteria must synthesise folic acid because they do not have a transporter. Without folic acid, bacteria cannot grow and divide. Therefore, because of sulfa drugs’ competitive inhibition, they are excellent antibacterial agents.
Non-competitive inhibitors
Non-competitive inhibitors bind to the enzyme at a different location, causing the active site to change shape and prevent the substrate from binding to it. During non-competitive inhibition, the enzyme may form complexes with either the substrate, the inhibitor, or both. Allosteric inhibition is non-competitive inhibition because the inhibitor binds to the allosteric site rather than the active site. Binding to an allosteric site causes the enzyme’s 3-dimensional tertiary structure to be distorted, preventing it from catalysing the process.
Because they effectively denature the enzymes, some non-competitive inhibitors are irreversible and permanent.
Pyruvate kinase catalyses the conversion of accumulated phosphoenol to pyruvate in the enzyme-catalysed processes of glycolysis. The amino acid alanine, produced from pyruvate, inhibits the enzyme pyruvate kinase during glycolysis. Alanine is a non-competitive inhibitor; it binds to the substrate away from the active site, allowing it to remain the end product.
Uncompetitive inhibition
Uncompetitive inhibition is a rare occurrence. An uncompetitive inhibitor binds to the enzyme and increases the substrate’s binding affinity, but the resulting enzyme-inhibitor-substrate complex reacts slowly to create the product. Note that before binding to the inhibitor, uncompetitive inhibition necessitates the creation of an enzyme-substrate complex.
Factors Affecting Enzyme Activity - Key takeaways
- Enzymes are big protein molecules that act as catalysts. They accelerate chemical reactions within the cell.
- Most enzymes are proteins that work to minimise the energy required to activate chemical reactions. They work with reactants called substrates.
- When the enzyme concentration increases, the reaction rates increase accordingly.
- When the substrate concentration increases, the reaction rate is only increased to a certain degree.
- High temperatures (above the optimum) will cause the enzyme’s linkages to break and the active site to lose its structure. It is denatured.
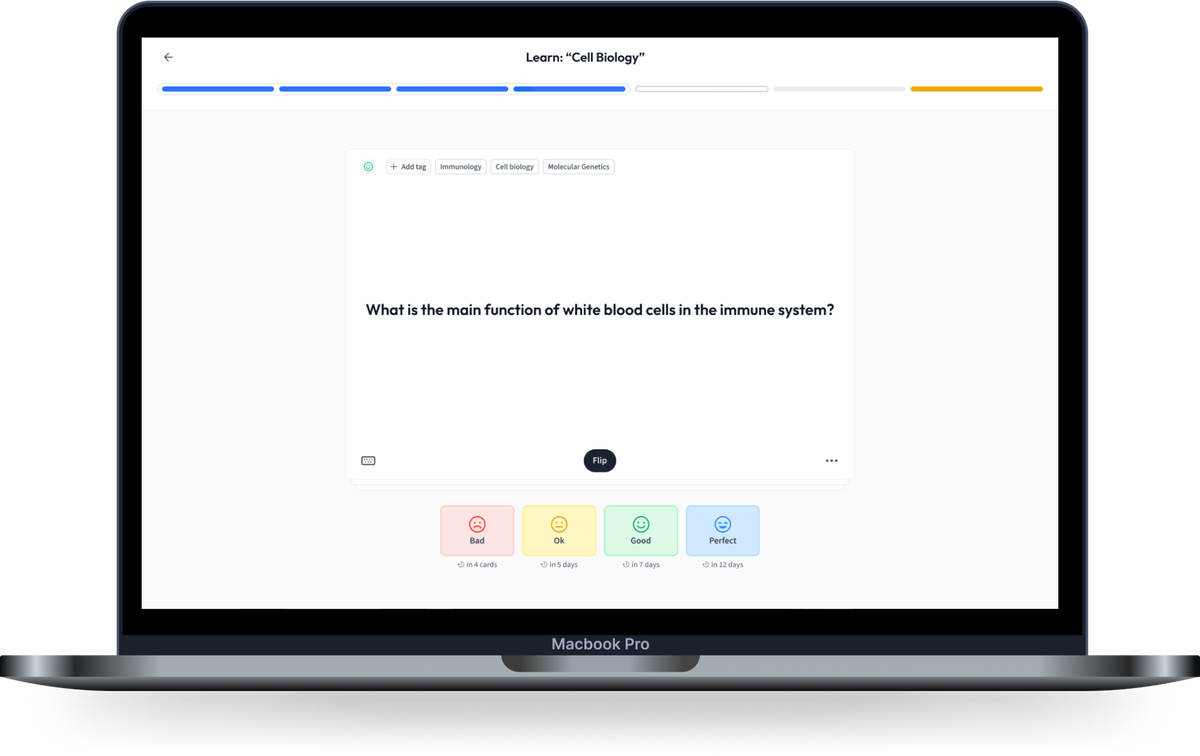
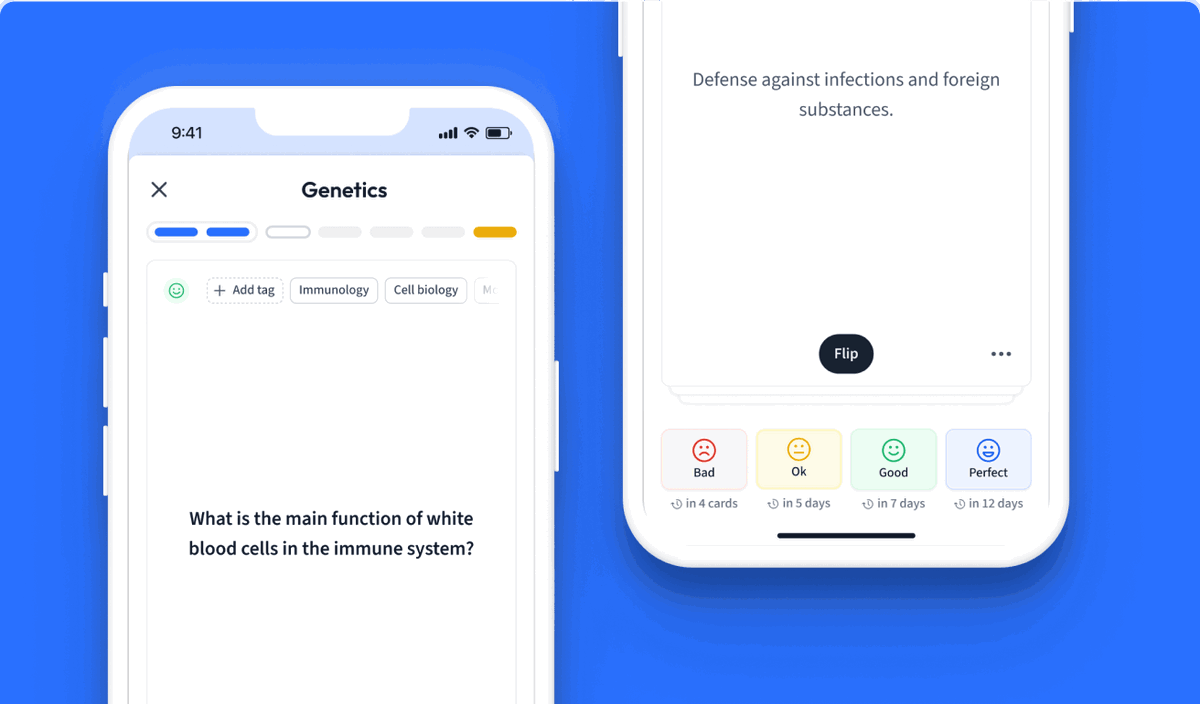
Learn with 0 Factors Affecting Enzyme Activity flashcards in the free StudySmarter app
We have 14,000 flashcards about Dynamic Landscapes.
Already have an account? Log in
Frequently Asked Questions about Factors Affecting Enzyme Activity
What factors affect enzyme activity?
Example factors are:
- Enzyme concentration
- Substrateconcentration
- Temperature
- pH
How does ph affect enzyme activity?
Each enzyme has a certain optimal pH at which the reaction rate is the fastest . A bell-shaped curve emerges when enzyme activity is plotted versus pH.
How does temperature affect enzyme activity?
The rate of an enzyme-catalyzed reaction increases as the temperature rises, as it does with many chemical reactions. At high temperatures, however, the rate drops again because the enzyme becomes denatured and no longer functions.
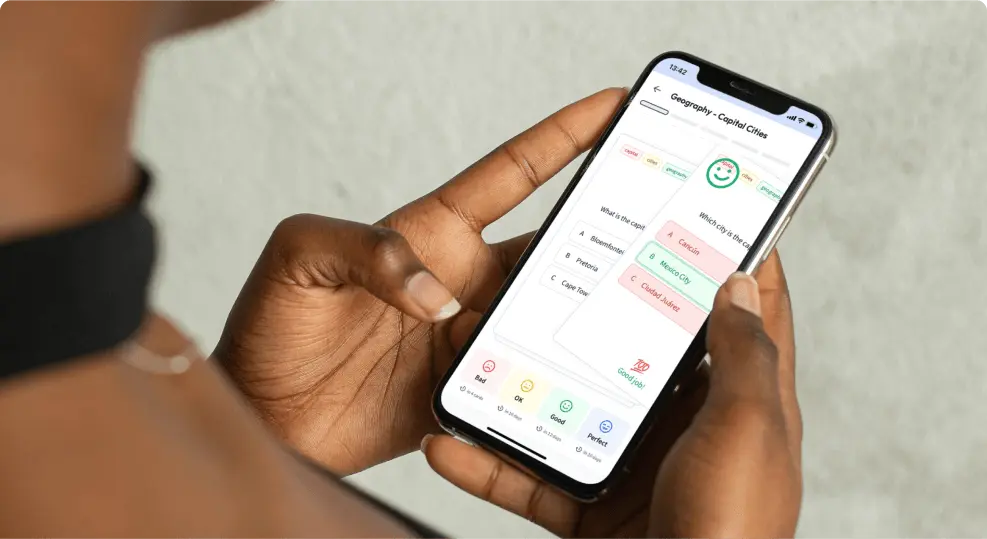
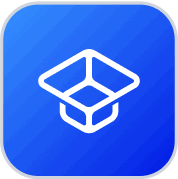
About StudySmarter
StudySmarter is a globally recognized educational technology company, offering a holistic learning platform designed for students of all ages and educational levels. Our platform provides learning support for a wide range of subjects, including STEM, Social Sciences, and Languages and also helps students to successfully master various tests and exams worldwide, such as GCSE, A Level, SAT, ACT, Abitur, and more. We offer an extensive library of learning materials, including interactive flashcards, comprehensive textbook solutions, and detailed explanations. The cutting-edge technology and tools we provide help students create their own learning materials. StudySmarter’s content is not only expert-verified but also regularly updated to ensure accuracy and relevance.
Learn more